|
- DNA repair in Chromobacterium violaceum
- Fábio Teixeira Duarte, Fabíola Marques de Carvalho, Uaska Bezerra e Silva,
- Kátia Castanho Scortecci, Carlos Alfredo Galindo Blaha, Lucymara Fassarella Agnez-Lima
- and Silvia Regina Batistuzzo de Medeiros
- Departamento de Biologia Celular e Genética, Centro de Biociências,
- Universidade Federal do Rio Grande do Norte, Campus Universitário,
- Lagoa Nova, 59072-970 Natal, RN, Brasil
- Corresponding author: S.R.B. Medeiros
- E-mail: [email protected]
- Genet. Mol. Res. 3 (1): 167-180 (2004)
- Received October 13, 2003
- Accepted January 12, 2004
- Published March 31, 2004
ABSTRACT. Chromobacterium violaceum is a Gram-negative b-proteobacterium that inhabits a variety of ecosystems in tropical and subtropical regions, including the water and banks of the Negro River in the Brazilian Amazon. This bacterium has been the subject of extensive study over the last three decades, due to its biotechnological properties, including the characteristic violacein pigment, which has antimicrobial and anti-tumoral activities. C. violaceum promotes the solubilization of gold in a mercury-free process, and has been used in the synthesis of homopolyesters suitable for the production of biodegradable polymers. The complete genome sequence of this organism has been completed by the Brazilian National Genome Project Consortium. The aim of our group was to study the DNA repair genes in this organism, due to their importance in the maintenance of genomic integrity. We identified DNA repair genes involved in different pathways in C. violaceum through a similarity search against known sequences deposited in databases. The phylogenetic analyses were done using programs of the PHILYP package. This analysis revealed various metabolic pathways, including photoreactivation, base excision repair, nucleotide excision repair, mismatch repair, recombinational repair, and the SOS system. The similarity between the C. violaceum sequences and those of Neisserie miningitidis and Ralstonia solanacearum was greater than that between the C. violaceum and Escherichia coli sequences. The peculiarities found in the C. violaceum genome were the absence of LexA, some horizontal transfer events and a large number of repair genes involved with alkyl and oxidative DNA damage.
Key words: DNA repair, Chromobacterium violaceum, Free-living organism, Genome
INTRODUCTION
Chromobacterium violaceum is a Gram-negative b-proteobacterium belonging to the Neisseriaceae family (Garrity et al., 2001). It is a free-living organism, with facultative anaerobic respiration, found in tropical and subtropical regions, including the water and banks of the Negro River, the largest tributary of the Amazon River (Caldas, 1990).
Although C. violaceum is considered a saprophyte, it can be an extremely virulent opportunistic pathogen for animals and humans (Hodge, 2002; Perera et al., 2003; Chen et al, 2003). An important characteristic of this organism is a purple pigment, known as violacein, responsible for many antimicrobial activities. It has a very potent bactericidal activity and can also be employed against Trypanosoma cruzi as well as against Mycobacterium tuberculosis and Leishmania sp. In addition, it has anti-viral and antitumoral properties. A review of C. violaceum can be found in Durán and Menck (2001).
Besides these medical applications, C. violaceum also has other biotechnological uses. It can be used for the extraction of gold from soil, as a result of the production of cyanide, which reacts with gold in order to form a complex anion [Au(CN)2]-; then gold is solubilized and easily extracted. This system could become very important for environmental detoxification and for improving the efficiency of mineral extraction (Campbell et al., 2001). It produces some polyhydroxyalkonoates, which might be an alternative to petrochemical plastic (Campbell et al., 2001). Chromobacterium violaceum also has potential for agricultural use, since it produces chitinases against insects, fungi and nematodes (Cronin et al., 1997; Patil et al., 2000).
Due to these remarkable qualities and because, among the few other free-living bacteria that have had their genomes sequenced, C. violaceum is one of the few that does not live in extreme conditions, the Brazilian National Genome Project Consortium decided to determine the sequence of its entire genome (Vasconcelos et al., 2003).
The survival of an organism is directly affected by its genetic stability, which depends on efficient replication or on DNA repair processes. In fact, DNA is constantly exposed to damaging agents, both endogenously from hydrolysis and oxidation, and exogenously of different kinds of physical (UV light, ionizing radiation), chemical and biological origins (Friedberg, 2003). These damages include DNA strand breaks and base loss, which creates an abasic site, and chemical modification of bases to form a mis-coding, or non-coding lesion. These damages may inhibit essential cellular processes, such as DNA replication or transcription, inducing cell death and/or mutations. In order to prevent this, cells have developed several different repair processes for removing most types of damage from DNA, or have developed processes for tolerating unexcised DNA damage when the genome is replicated (Friedberg, 2003). These processes include: i) repair by damage reversion, in which only one enzyme is necessary to directly reverse the damage; ii) mismatch repair, in which the enzymes involved are responsible for the correction of mismatched base pairs, mainly those induced by replication errors; iii) recombination repair or double-strand break repair, mediated by enzymes involved with homologous recombination, which use the information of undamaged sister chromatin or homologous regions to bypass lesions, including DNA strand breaks; iv) base excision repair, involving the action of glycosylases, which cleave the glycosydic bound between the specific damaged base and the deoxyribose, with subsequent incision by AP-endonuclease at the resultant abasic site; vi) nucleotide excision repair enzymes, which are able to remove several types of DNA damage, especially those that induce large double-helix distortion, and vii) SOS response, which is a cascade of cellular reactions induced by the activation of a large number of genes when the cell is exposed to the harmful action of DNA-damaging agents. These cellular responses are under the control of the transcriptional repressor LexA and are susceptible to error-prone replication (Goodman, 2002).
We identified genes involved in DNA repair pathways in C. violaceum through a similarity search against known sequences deposited in genetic databases. This search revealed the presence of all pathways similar to that found in E. coli but, as expected, the best e-values were obtained with DNA repair proteins from Neisseria meningitidis and Ralstonia solanacearum, species from the same family as C. violaceum.
MATERIAL AND METHODS
The C. violaceum genome was totally sequenced by the Brazilian National Genome Project Consortium (Vasconcellos et al., 2003). Open-reading frames from C. violaceum were compared with known DNA repair genes present in the GeneBank
(http://www.ncbi.nlm.nih.gov). A basic local alignment sequence tool (BLAST) was used for similarity searches against nucleotide and protein databases (Altschul et al., 1997). An e-value of less than 1 x 10-10 was used as a threshold of similarity for these searches.
Phylogenetic Analysis
Alignments of amino acid sequences from DNA repair-related sequences were made using the Clustal W program
(www.infobiogen.fr). The edition of the resulting alignment was done with the BioEdit program (Hall, 1999), prior to performing a phylogenetic analysis using the Phylip program (Felsenstein et al., 1989). The same phylogenetic analysis was done with 16S RNA sequences of several bacteria, for comparison. The taxonomic classification was based on the Bersgey’s Manual of Systematic Bacteriology (Garrity et al., 2001). Parsimony analysis was done by the Protpars and DNApars (Dayhoff and Orcutt, 1979). The tree was visualized with the Treview program (Page, 1996) and the consistency of the tree was determined by Bootstrap values from 1000 replicates (Zharkikh and Li, 1995).
RESULTS AND DISCUSSION
Damage Reversion
In principle, reversal of the lesion is the simplest mechanism by which damaged DNA might be repaired. The biochemical mechanism is based on a one-step reaction, where one specific enzyme recognizes and reverses the lesion to its normal configuration, in an error-free manner. Differently from other repair mechanisms, these enzymes do not participate in the same reaction. Thus, each enzyme reacts with different substrates and has different evolutionary origins (Eisen and Hanawalt, 1999). Photoreactivation and alkylation repair are two of the most well-studied reversal mechanisms. The former is a light-dependent pathway that acts upon lesions induced by UV irradiation (cyclobutane pyrimidine dimers and 6-4 photoproduct). This pathway is carried out by an enzyme, called photolyase, widely distributed among prokaryotes and eukaryotes. The latter is done by alkyltransferases, which are able to recognize alkyl, methyl and ethyl radicals covalently linked to DNA bases (Labahn et al., 1996). These proteins (Ogt and Ada) carry out alkylation reversal by transferring the alkyl group from the DNA to themselves, in a suicidal process (Labahn et al., 1996). The enzymes related to this pathway were determined for C. violaceum (Table 1). There is one copy of the photoreactivation gene (phrB) and two copies of the alkylation genes (ogt and ada). Since this bacterium is a free-living organism, which is constantly exposed to UV radiation, as well as to various dangerous compounds present in the natural environment, it is apparent that this pathway is an important protective mechanism, as is the duplication of some genes involved with the direct reversal pathway. The Ogt2 copy gave the best e-value against Gram-positive bacteria, suggesting a horizontal transference event. In order to determine how this event might have occurred, we looked more carefully at this sequence and we found that the ogt2 gene is near transposase sequences (Figure 1), which may mean that the ogt2 copy was inserted into the C. violaceum genome by transposition. Other supporting evidence is that the GC content of ogt2 is higher (72.37%) than that of the whole C. violaceum genome (64%). In addition, the two ogt copies are positioned far apart in the C. violaceum genome. As these duplications were not found in R. solanacearum and N. meningitidis, it is possible that this acquisition is a recent event, and not an ancient duplication in the b-proteobacterium genome, followed by a gene deletion.
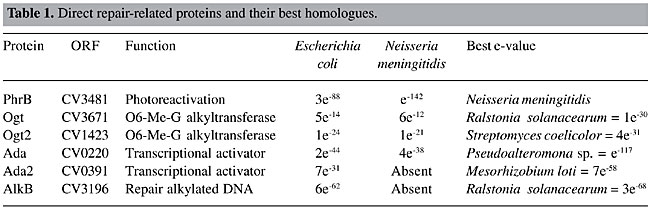
Figure 1. Localization of the ogt2 gene in the Chromobacterium violaceum genome.
Base Excision Repair
Base excision repair recognizes a wide range of DNA lesions, such as imidazole ring-opened, deaminated, alkylated, oxidized bases and abasic sites. This pathway is essentially run by two kinds of enzymes: glycosylases and AP-endonucleases. The former recognizes and excises the damaged base from the sugar phosphate backbone, generating an abasic site (AP), and the latter makes an incision at the 5’or 3’ phosphodiester from the AP site, generating a nucleotide gap. A DNA polymerase fills then this gap, and the DNA sequence is restored by DNA ligase (Mol et al., 1999). There are a large number of glycosylases and AP-endonucleases in the C. violaceum genome (Table 2), indicating that this organism is able to repair various kinds of base damage.
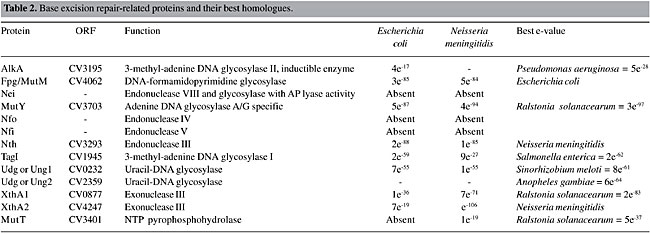
Uracil DNA glycosylase (UNG) is the main enzyme involved in the insertion of uracil. In DNA, this base results from a cytosine deamination, which gives rise to G:U mismatches, leading to G:C to A:T transition mutations during replication. Another uracil source in DNA is the incorporation of dUMP, instead of dTMP. The U:A pairs are not mutagenic, but they may alter the binding of transcription factors to their target, affecting gene-expression (Mol et al., 1999). UNG homologues have been characterized in many bacterial and eukaryotic species, and these proteins have strikingly similar structures and functions (Pearl, 2000). In contrast to the proteobacteria group, C. violaceum has two copies of this gene. The alkylglycosylases can be divided into two groups in prokaryotes: AlkA, which are inductible and TagI, which are constitutive. The formamidopyrimidine DNA glycosylase (FPG or MutM) and MutY-glycosylase, important enzymes for oxidative repair, are also present in the C. violaceum genome. The former recognizes oxidized purines, such as 7,8-dihydro-8-deoxyguanine (8-oxo-dG) and imidazole ring-opened purines (FAPY-G), and the latter removes the deoxyadenine, when paired with 8-oxo-dG, deoxyguanosine or deoxycytosine (Fowler et al., 2003). Together with MutT enzyme, these two enzymes belong to the GO system, which protects the cell against the effects of the oxidative stress product, 8-oxoguanine (8-oxoG), a base with ambiguous base-pairing properties, pairing with either A or C during DNA synthesis (Fowler et al., 2003). The GO system is complete in the C. violaceum genome, as the MutT protein is present in the CV1787 open-reading frame.
Nucleotide Excision Repair
Nucleotide excision repair is the most general DNA repair pathway, as it recognizes all lesions that generate a large helical distortion (Batty and Wood, 2000). Its mechanisms consist of the following four steps: a) recognition of the damaged DNA; b) excision of a 24-32-residue oligonucleotide, containing the DNA damage, by dual incision of the damaged strand on each side of the lesion; c) filling in of the resulting gap by DNA polymerase, and d) ligation of the nick (Batty and Wood, 2000). Although there is a high similarity of this pathway between prokaryotes and eukaryotes, their evolutionary origins are different (Eisen and Hanawalt, 1999). The enzymes involved in this pathway in C. violaceum were determined (Table 3). All the enzymes are present and they had the highest e-values, suggesting that this pathway is the most conserved among all DNA repair pathways. These genes were also found to be highly conserved by Eisen and Hanawalt (1999). We suggest that these genes could be used as new bioinformatics tools for phylogenetic analysis.
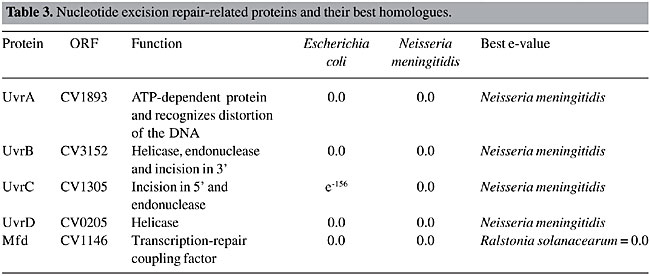
Mismatch Repair
DNA mismatch repair is a major contributor to DNA replication fidelity by removing insertion/deletion loops that result from template primer slippage at repetitive DNA sequences and by correcting single-base mismatches that escape polymerase proofreading (Marti et al., 2002). This process is well characterized in E. coli, and it can be divided into five steps: 1) recognition of the DNA lesion by the MutS homodimer; 2) interaction of the mismatch-bound MutS homodimer with both ATP and the MutL homodimer, resulting in activation of the MutS-MutL complex; 3) identification of the nascent DNA by MutH endonuclease, through the recognition of hemimethylation at GATC sequences; 4) removal of up to 1000 bases in the nascent DNA surrounding the lesion by processes involving MutH endonuclease, MutU (also known as UvrD and DNA helicase II), and exonucleases specific for single-strand DNA, and 5) resynthesis of DNA by the replicative PolIII holo-enzyme. The genes found in C. violaceum genome that participate in this pathway have been determined (Table 4). The most important enzymes of this pathway are present. However, the enzyme (Dam) involved in DNA methylation, the signal of mismatch repair, was found to have a high similarity to a bacteriophage enzyme, suggesting a horizontal transfer event. To better understand this situation, a phylogenetic tree was constructed, using parsimony (Figure 2) with the 16S RNA tree (Figure 2A) as a tree model, since it is well established for phylogenetic analysis, and the Dam tree (Figure 2B). The phylogenetic trees clearly show the relationship between Dam sequences of C. violaceum and bacteriophages. It seems that C. violaceum bacteria have lost the bacterial Dam but, as this sequence is important for many cell functions (Urig et al., 2002), it subsequently acquired this gene, probably by transduction.
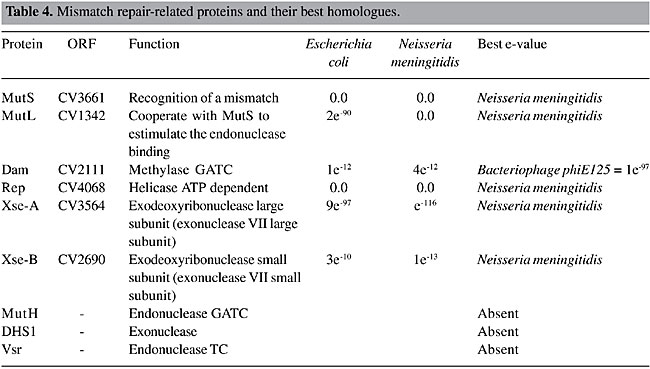
Figure 2. Phylogenetic unrooted tree of 16S RNA (A) and Dam methylases (B) from Chromobacterium violaceum and several bacteriophages. The tree was constructed using parsimony, with 1000 replicates.
Recombinational Repair
The recombinational repair pathway is activated, mainly when double-strand breaks are generated. If not repaired, double-strand breaks result in broken chromosomes, and finally in cell death. In prokaryotes, this damage is repaired by homologous recombination, which has the potential to make an accurate repair. Homologous recombination can be divided into four steps: a) initiation; b) strand pairing and exchange; c) branch migration, and d) branch resolution. Different pathways within a species often differ from each other in the first and the last steps, but they use the same mechanism for the pairing and exchange step (Cromie et al., 2001). C. violaceum has only one pathway for the initiation (RecBCD) and two for branch migration and resolution (RuvABC and RecG, Table 5). RecA is universally found in bacteria, and the C. violaceum genome is not an exception. The same organization for this kind of DNA repair has been found in other proteobacteria (Eisen and Hanawalt, 1999). Eisen (1995) found that RecA proteins have some conserved regions among species, while other regions are highly variable; they are informative for studies of molecular systematics of bacteria. The phylogenetic tree generated with RecA peptide sequences has the same pattern of organism distribution as those made with 16S RNA (data not shown), indicating that this sequence would be useful as a tool for phylogenetic analysis.
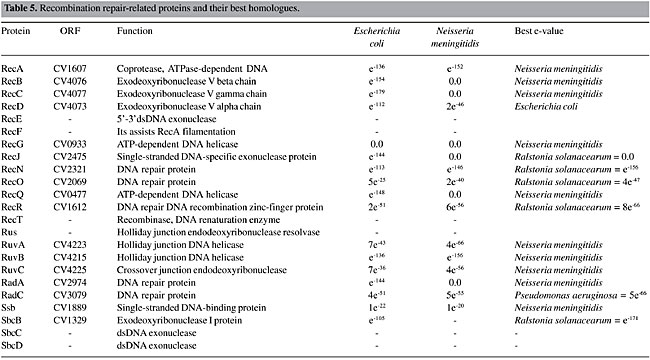
SOS Response
When DNA is damaged, replication is blocked, and several genes responsible for the elaboration of a set of physiological responses, such as inhibition of the cell cycle, induction of several DNA repair pathways, including nucleotide excision repair, recombinational repair and error-prone translesion repair, are activated (Sutton et al., 2000). The SOS response is controlled by a dual-component system, in which RecA protein is the activator and LexA the repressor. In response to DNA damage, RecA protein nucleates on single-stranded DNA. This complex acts to mediate the cleavage of LexA repressor, leading to the expression of the SOS regulon, a collection of more than 30 genes, including recA and lexA. Among these genes, there are some polymerases involved with error-prone Pol II (polB), Pol IV (dinB) and Pol V (umuDC) translesion syntesis. However, depending upon the nature of the DNA damage, and its sequence context, Pol II and Pol IV can also be involved in error-free translesion synthesis (Napolitano et al., 2000). The genes related to the SOS response in C. violaceum have been identified (Table 6). The absence of LexA protein can be observed. As LexA is important for the inducibility of this system, the lexA regulatory box was searched for throughout the C. violaceum genome, but no match was found. This might indicate a constitutive SOS response in the C. violaceum genome, or it could be regulated by another, yet unknown, protein. Among the b-proteobacteria, only R. solanacearum has the lexA gene, which suggests a recent loss of this gene in the other b-proteobacteria (C. violaceum, Neisseria gonorrhoeae and N. miningitidis). It is known that the recA, uvrA and uvrB genes from N. gonorrhoeae lack a characteristic lexA-binding site in the promoter region (Fyfe and Davies, 1990; Black et al., 1997). Black et al. (1998) made a Northern dot blot hybridization analysis to determine if there was an SOS-like system, but he failed to find any SOS response, suggesting a constitutive pathway in N. gonorrhoeae. Since C. violaceum is a free-living organism, constantly exposed to damage, and since it belongs to the same family as N. gonorrhoeae, it is possible that the SOS is also constitutive. However, functional analysis must be done to clarify this hypothesis. Among the DNA polymerases induced by the SOS system, we find the umuC and umuD genes, components of Pol V and Pol IV; these are translesion polymerases.
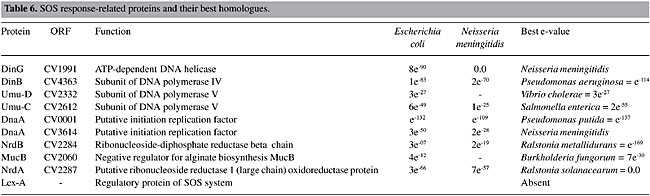
CONCLUDING REMARKS
DNA repair mechanisms are well conserved among living organisms, due to their importance in maintaining genome integrity. The cells are provided with different DNA repair pathways in accordance with their adaptability. C. violaceum is a free-living organism, belonging to the b-proteobacteria, the genomic organization of which is not well characterized. The search for components of the many DNA repair pathways in this bacterium, revealed the great versatility of this organism, as all the pathways are present. Moreover, the C. violaceum sequences displayed a higher similarity to N. meningitidis and to R. solanacearum proteins than to E. coli proteins. This is not a surprising result as these organisms belong to the same class, despite their different characteristics. C. violaceum is a free-living organism, present in sand banks and waters of tropical rivers, and it rarely infects animals and human beings. N. meningitidis causes bacterial meningitis, and it is responsible for considerable morbidity and mortality in both the developed and the under-developed world. Meningococci are opportunistic pathogens that colonize the nasopharynges and oropharynges of asymptomatic carriers. Little is known about their mechanism of action, but they occasionally gain access to the blood, and subsequently to the cerebrospinal fluid, causing septicemia (Parkhill et al., 2000). R. solanacearum is a devastating, soil-borne plant pathogen, with a global distribution and an unusually wide host range (Salanoubat et al., 2002).
The most interesting feature observed in the C. violaceum genome is the presence of a large number of duplicated genes (ogt, ada, ung, xth), suggesting a necessity for a more elaborate defense against alkylation and oxidative DNA damages. Aside from the ogt and ada duplications, the alkA and tagI genes are also present in the C. violaceum genome. Several copies of tag genes have been observed in plant genomes, like Arabidopsis thaliana (Hays, 2002), sugarcane and Oriza sativa (Agnez-Lima et al., 2001; Scortecci, K.C., Lima, A.F.O., Azevedo, A.K.N., Cardoso, C.Q., Araújo, K.M., Bezerra, M.E.T., Silva, R.C., Silva, U.B., Batistuzzo de Medeiros, S.R. and Agnez-Lima, L.F., personal communication). Plants and free-living organisms are constantly exposed to a large number of DNA damaging agents. Considering the presence of the aforementioned duplicated genes, it is possible that alkylation agents are abundant in the environment. These genes might have an important adaptive advantage. Two events of horizontal transfer were identified: one by transposition (ogt) and the other by transduction (dam methylase).
ACKNOWLEDGMENTS
The C. violaceum genome sequencing project was supported by funds from the MCT/CNPq in the Brazilian National Genome Project Consortium. Research also supported by the Secretaria da Indústria, do Comércio, da Ciência e da Tecnologia do Estado do Rio Grande do Norte. F.T. Duarte received fellowship from CAPES. C.A.G. Blaha and K.C. Scortecci were recipients of post-doctoral fellowships from CNPq.
REFERENCES
Agnez-Lima, L.F., Batistuzzo de Medeiros, S.R., Maggi, B.S. and Quaresma, G.A.S. (2001). Base excision repair. Genet. Mol. Biol. 24: 123-129.
Altschul, S.F., Madden, T.L., Schäffer, A.A., Zhang, J., Zhang, Z., Miller, W. and Lipman, D.J. (1997). Gapped BLAST and PSI-BLAST: a new generation of protein database search programs. Nucleic Acids Res. 25: 3389-3402.
Batty, D.P. and Wood, R.D. (2000). Damage recognition in nucleotide excision repair of DNA. Gene 241: 193-204.
Black, C.G., Fyfe, J.A. and Davies, J.K. (1997). Cloning, nucleotide sequence and transcriptional analysis of the uvrA gene from Neisseria gonorrhoeae. Mol. Gen. Genet. 254: 479-485.
Black, C.G., Fyfe, J.A. and Davies, J.K. (1998). Absence of an SOS-like system in Neisseria gonorrhoeae. Gene 208: 61-66.
Caldas, L.R. (1990). Um pigmento nas águas negras. Cienc. Hoje 11: 55-57.
Campbell, S.C., Olson, G.J., Clark, T.R. and McFeters, G. (2001). Biogenic production of cyanide and its application to gold recovery. J. Ind. Microbiol. Biotechnol. 26: 134-139.
Chen, C.H., Lin, L.C., Liu, C.E. and Young, T.G. (2003). Chromobacterium violaceum bacteremia: a case report. J. Microbiol. Immunol. Infect. 36: 141-144.
Cromie, G.A., Connelly, J.C. and Leach, D.R. (2001). Recombination at double-strand breaks and DNA ends: conserved mechanisms from phage to humans. Mol. Cell. 8: 1163-1174.
Cronin, D., Moenne-Loccoz, Y., Dunne, C. and O´Gara, F. (1997). Inhibition of egg hatch of the potato cyst nematode Globodera rostochiensis by chitinase-producing bacteria. Eur. J. Plant Pathol. 103: 433-440.
Dayhoff, M.O. and Orcutt, B.C. (1979). Methods for identifying proteins by using partial sequences. Proc. Natl. Acad. Sci. USA 76: 2170-2174.
Durán, N. and Menck, C.F.M. (2001). Chromobacterium violaceum: a review of pharmacological and industrial perspectives. Crit. Rev. Microbiol. 3: 201-222.
Eisen, J.A. (1995). The RecA protein as a model molecule for molecular systematic studies of bacteria: comparison of trees of RecAs and 16S rRNAs from the same species. J. Mol. Evol. 41: 1105-1123.
Eisen, J.A. and Hanawald, P.C. (1999). A phylogenomic study of DNA repair genes, proteins, and processes. Mutat. Res. 435: 171-213.
Felsenstein, J. (1989). PHYLIP Phylogeny Inference Package (3.2). Cladistics 5: 164-166.
Fowler, R.G., White, S.J., Koyama, C., Moore, S.C., Dunn, R.L. and Schaaper, R.M. (2003). Interactions among the Escherichia coli mutT, mutM, and mutY damage prevention pathways. DNA Repair 2: 159-173.
Friedberg, E.C. (2003). DNA damage and repair. Nature 421: 436-440.
Fyfe, J.A. and Davies, J.K. (1990). Nucleotide sequence and expression in Escherichia coli of the recA gene of Neisseria gonorrhoeae. Gene 93: 151-156.
Garrity, G.M., Winters, M. and Searles, D.B. (2001). Taxonomic outline of the prokaryotic genera, Bergey’s Manual of Systematic Bacteriology. 2nd edn. Springer-Verlag, New York, NY, USA, pp. 1-39.
Goodman, M.F. (2002). Error-prone repair DNA polymerases in prokaryotes and eukaryotes. Annu. Rev. Biochem. 71: 17-50.
Hall, T.A. (1999). BioEdit: a user-friendly biological sequence alignment editor and analysis program for Windows 95/98/NT. Nucleic Acids Symp. Ser. 41: 95-98.
Hays, J.B. (2002). Arabidopsis thaliana, a versatile model system for study of eukaryotic genome-maintenance functions. DNA Repair 1: 579-600.
Hodge, R.A. (2002). Non-chromogenic Chromobacterium violaceum in a urinary tract infection. Clin. Microbiol. Newsl. 24: 15 (Abstract).
Labahn, J., Scharer, O.D., Long, A., Ezaz-Nikpay, K., Verdine, G.L. and Ellenberger, T.E. (1996). Structural basis for the excision repair of alkylation-damage DNA. Cell 86: 321-329.
Marti, T.M., Kunz, C. and Fleck, O. (2002). DNA mismatch repair and mutation avoidance pathways. J. Cell. Physiol. 191: 28-41.
Mol, C.D., Parikh, S.S., Putnam, C.D., Lo, T.P. and Tainer, J.A. (1999). DNA repair mechanisms for the recognition and removal of damaged DNA bases. Annu. Rev. Biophys. Biomol. Struct. 28: 101-128.
Napolitano, R, Janel-Bintz, R., Wagner, J. and Fuchs, R.P. (2000). All three SOS-inducible DNA polymerases (Pol II, Pol IV and Pol V) are involved in induced mutagenesis. EMBO J. 19: 6259-6265.
Page, R.D. (1996). TreeView: an application to display phylogenetic trees on personal computers. Comput. Appl. Biosci. 12: 357-358.
Parkhill, J., Achtman, M., James, K.D., Bentley, S.D., Churcher, C., Klee, S.R., Morelli, G., Basham, D., Brown, D., Chillingworth, T., Davies, R.M., Davis, P., Devlin, K., Feltwell, T., Hamlin, N., Holroyd, S., Jagels, K., Leather, S., Moule, S., Mungall, K., Quail, M.A., Rajandream, M.A., Rutherford, K.M., Simmonds, M., Skelton, J., Whitehead, S., Spratt, B.G. and Barrell, B.G. (2000). Complete DNA sequence of a serogroup A strain of Neisseria meningitidis Z2491. Nature 404: 502-506.
Patil, R.S., Ghormade, V. and Despande, M.V. (2000). Chitinolytic enzymes: an exploration. Enzyme Microb. Technol. 26: 473-483.
Pearl, L.H. (2000). Structure and function in the uracil-DNA glycosylase superfamily. Mut. Res. 460: 165-181.
Perera, S., Punchihewa, P.M., Karunanayake, M.C. and De Silva, N. (2003). Fatal septicemia caused by Chromobacterium violaceum. Ceylon Med. J. 48: 26-27.
Salanoubat, M., Genin, S., Artiguenave, F., Gouzy, J., Mangenot, S., Arlat, M., Billault, A., Brottier, P., Camus, J.C., Cattolico, L., Chandler, M., Choisne, N., Claudel-Renard, C., Cunnac, S., Demange, N., Gaspin, C., Lavie, M., Moisan, A., Robert, C., Saurin, W., Schiex, T., Siguier, P., Thebault, P., Whalen, M., Wincker, P., Levy, M., Weissenbach, J. and Boucher, C.A. (2002). Genome sequence of the plant pathogen Ralstonia solanacearum. Nature 415: 497-502.
Sutton, M.D., Smith, B.T., Godoy, V.G. and Walker, G.C. (2000). The SOS response: recent insights into umuDC-dependent mutagenesis and DNA damage tolerance. Annu. Rev. Genet. 34: 479-497.
Urig, S., Gowher, H., Hermann, A., Beck, C., Fatemi, M., Humeny, A. and Jeltsch, A. (2002). The Escherichia coli dam DNA methyltransferase modifies DNA in a highly processive reaction. J. Mol. Biol. 319: 1085-1096.
Vasconcelos, A.T.R., Almeida, D.F., Almeida, F.C., Almeida, L.G.P., Almeida, R., Alves-Gomes, J.A., Andrade, E.M., Antônio, R.V., Araripe, J., Araújo, M.F.F., Astolfi-Filho, S., Azevedo, V., Baptista, A.J., Bataus, L.A.M., Batista, J.S., Beló, A., van den Berg, C., Blamey, J., Bogo, M., Bonatto, S., Bordignon, J., Brito, C.A., Brocchi, M., Burity, H.A., Camargo, A.A., Cardoso, D.D.P., Carneiro, N.P., Carraro, D.M., Carvalho, C.M.B., Cascardo, J.C.M., Cavada, B.S., Chueire, L.M.O., Creczynski-Pasa, T.B., Duran, N., Fagundes, N., Falcão, C.L., Fantinatti, F., Farias, I.P., Felipe, M.S.S., Ferrari, L.P., Ferro, J.A., Ferro, M.I.T., Franco, G.R., Freitas, N.S.A., Furlan, L.R., Gazzinelli, R.T., Gomes, E.A., Gonçalves, P.R., Grangeiro, T.B., Grattapaglia, D., Grisard, E.C., Guimarães, C.T., Hanna, E.S., Hungria, M., Jardim, S.N., Laurino, J., Leoi, L.C.T., Lima, L.F.A., Loureiro, M.F., Lyra, M.C.C.P., Macedo, M., Madeira, H.M.F., Manfio, G.P., Maranhão, A.Q., Martins, W.S., di Mauro, S.M.Z., Batistuzzo de Medeiros, S.R., Meissner, R.V., Moreira, M.A.M., Nascimento, F.F., Nicolas, M.F., Oliveira, J.G., Oliveira, S.C., Paixão, R.F.C., Parente, J.A., Pedrosa, F.O., Pena, S.D.J., Pereira, J.O., Pereira, M., Pinto, L.S.R.C., Pinto, L.S., Porto, J.I.R., Potrich, D.P., Ramalho-Neto, C.E., Reis, A.M.M., Rigo, L.U., Rondinelli, E., Santos, E.B.P., Santos, F.R., Schneider, M.P.C., Seuanez, H.N., Silva, A.M.R., Silva, A.L.C., Silva, D.W., Silva, R., Simões, I.D.C., Simon, D., Soares, C.M.A., Soares, R.B.A., Souza, E.M., Souza, K.R.L., Souza, R.C., Steffens, M.B.R., Steindel, M., Teixeira, S.R., Urmenyi, T., Vettore, A., Wassem, R., Zaha, A. and Simpson, A.J.G. (2003). Complete genome sequence of Chromobacterium violaceum reveals remarkable and exploitable bacterial adaptability. Proc. Natl. Acad. Sci. USA 100: 11660-11665.
Zharkikh, A. and Li, W.H. (1995). Estimation of confidence in phylogeny: the complete-and-partial bootstrap technique. Mol. Phylogenet. Evol. 4: 44-63.
|
|
|
|